Research Overview - Microfluidic and Surface Electrochemistry
Introduction
Fuels cells are an example of a device where surface electrochemistry plays a central role. Surface electrochemistry is the part of electrochemistry concerned with how the molecules interact with the surface. At an electrode in a fuel cell, a chemical reaction takes place, for example at the anode of a direct methanol fuel cell, the reaction is the oxidation of methanol to carbon dioxide:

As written, it appears that a methanol molecule and a water molecule move to the surface of the electrode, where they react in a single step and pass six electrons to the electrode. However, the actual process is more complicated than this and the surface of the electrode play an active and important role. The methanol and water react with the surface to give various intermediate molecules adsorbed on the surface. In a complicated series of reaction steps, these molecules interact and transform on the surface and then release the product molecules. The surface is an electrocatalyst and understanding the details of the reaction steps is crucial to improving the performance of the fuel cell. Some projects involve reactions other than fuel oxidations, for example we are interested in metal electrodeposition, oxide formation and peroxide reduction.
Direct Liquid Fuel Cells in a Sustainable Energy Future
Our main focus is on oxidation of small organic molecules. These can be the
fuels in a "direct" fuel cell, i.e., the fuel reacts directly at the electrode
surface. (The alternative, to convert the fuel in a reformer to hydrogen and use the hydrogen as the fuel
reacting at the electrode, leads to additional complexity and a loss of
efficiency.). Liquid fuels are easy to transport and have a higher energy
density than gaseous fuels. If the fuel is derived from atmospheric carbon
dioxide, for example by harvesting the fuel from a plant source, then the
overall cycle of production of the fuel and then its conversion back to
CO in a fuel cell leads to no net change in atmospheric
CO
levels, and does not contribute to global warming. Our focus is therefore on
molecules that can be derived from biomass; this includes formic acid,
methanol and ethanol. In this sequence, there are increasing catalytic
challenges. In formic acid, both oxygen atoms are already attached to carbon,
and the production of
CO
is easiest. Methanol catalysis has to bring the oxygen from a water molecule
to the carbon in methanol. In ethanol catalysis there is the additional
challenge of breaking a carbon-carbon bond.
Microfluidic Electrochemistry
We are interested in coupling microfluidics with electrochemistry, both to make microfluidic fuel cells and to use microfluidic methods to determine the reaction mechanism in the electrochemical oxidation of organic fuels. Microfluidics involves the flow of liquids in small devices, on the submillimetre scale. Fluid devices on this scale have some distinct benefits, which can be illustrated by considering a laminar flow fuel cell constructed around a channel with two electrodes on either side:
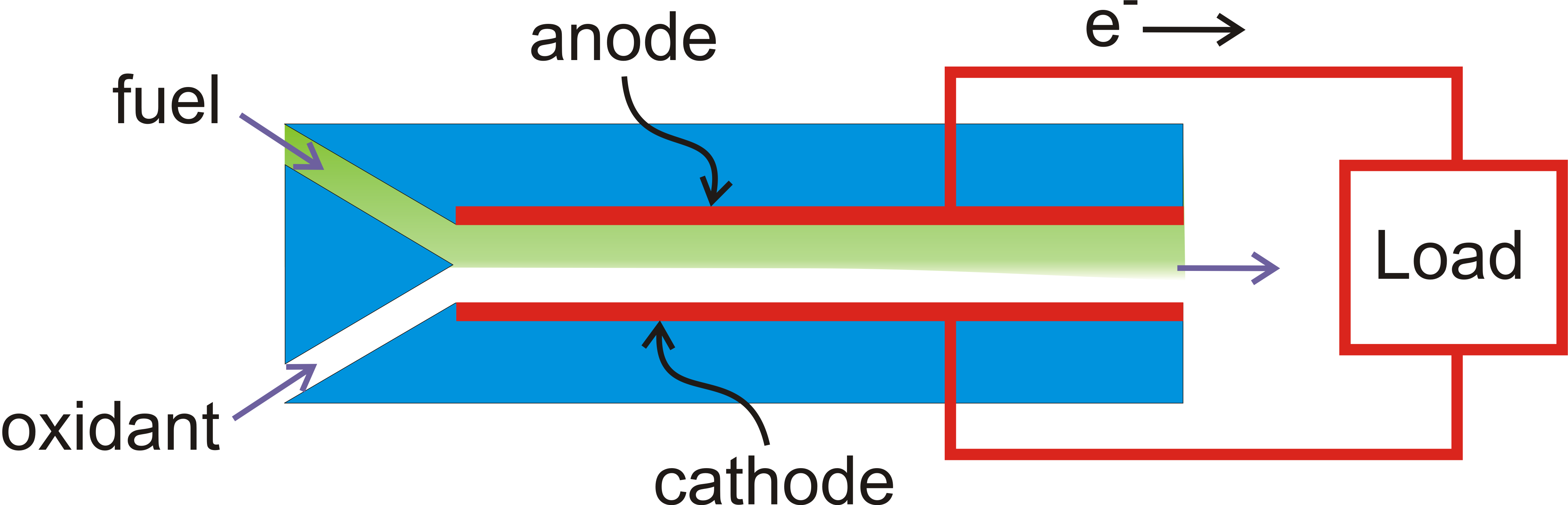
The fuel stream (e.g., methanol in sulfuric acid, green) and the oxidant stream (e.g., oxygen or hydrogen peroxide in sulfuric acid, white) flow from the left. Because of the small dimensions of the device, turbulence does not occur, and mixing of the green and white flows occurs only to a very limited extent. Therefore the fuel and oxidant are separated without the use of a membrane, which would be required in a conventional fuel cell. Furthermore, the thickness of the channel (top to bottom) is less that the thickness of the diffusion layer, so that the performance of the cell is not limited by diffusion of the reactants to the electrode. This means that the fuel utilization is high; no unused fuel will flow out and there is no need to recirculate fuel.
Methods
Conventional Electrochemical Methods
Electrochemical kinetic methods give kinetic information about the adsorption or reaction processes taking place. These methods are very sensitive (we can easily measure reactions of molecules comprising only a few percent of a monomolecular layer (monolayer)). They can be used to find rate constants and other mechanistic information. However, they do not unambiguously identify the nature of the species involved or the way they are bonded. We mostly use cyclic voltammetry, potential step measurements, and impedance methods (see below), but are equipped to carry out almost any conventional electrochemical method. We have many potentiostat and function generator setups, some supplemented with lockin-amplifiers, rotating disk electrodes (RDE), and rotating ring-disk electrodes (RRDE) or the quartz crystal microbalance. Data is acquired on digital oscilloscopes or digitizers and analyzed using commercial and custom-written software.
Electrochemical Quartz-Crystal Microbalance
The electrochemical quartz-crystal microbalance (EQCM) uses the change in resonance frequancy of an oscillating quartz crystal to measure mass changes accompanying electrochemical reactions. This allows us to deduce the identity of the species depositing at the electrode surface. Complications due to artifacts such as surface roughness and due to the presence of anions can complicate interpretation of this method. We are developing faster EQCM methods and the mass impedance method to allow us to decouple processes that occur together on the slower voltammetry time scale.
Electrochemical Impedance Spectroscopy
Electrochemical impedance spectroscopy (EIS) is a powerful method to extract rate constants for electrochemical processes. One limitation to the usual method is that reactions which do not achieve steady-state cannot be studied, e.g. an irreversible adsorption reaction which proceeds to completion of a monolayer and then stops. To catch the active adsorption region, we have implemented the dynamic EIS method (dEIS), with acquisition of data during a cyclic voltammetry scan. We have applied this method to study oxide growth on Pt, Tl UPD on iodine-covered Pt(111) and the oxidation of methanol and formic acid on Pt electrodes. Recently, we have upgraded the instrumentation to make use of Fourier transform methods, much decreasing the acquisition time. Selected EIS spectra around a formic acid voltammogram are shown below, and it is evident from the difference between spectra going forward (top spectra) and backward (bottom spectra) that the dynamic nature of the surface is being probed. The spectra show dramatic changes, sometimes over only 2-3 mV, demonstrating that they reveal the complexity of the mechanism that is hidden in the rather featureless voltammogram. For example, the flip across the axis in the sequence of three outlined spectra signals incipient oscillating behavior, and was used to find conditions that initiated oscillations.
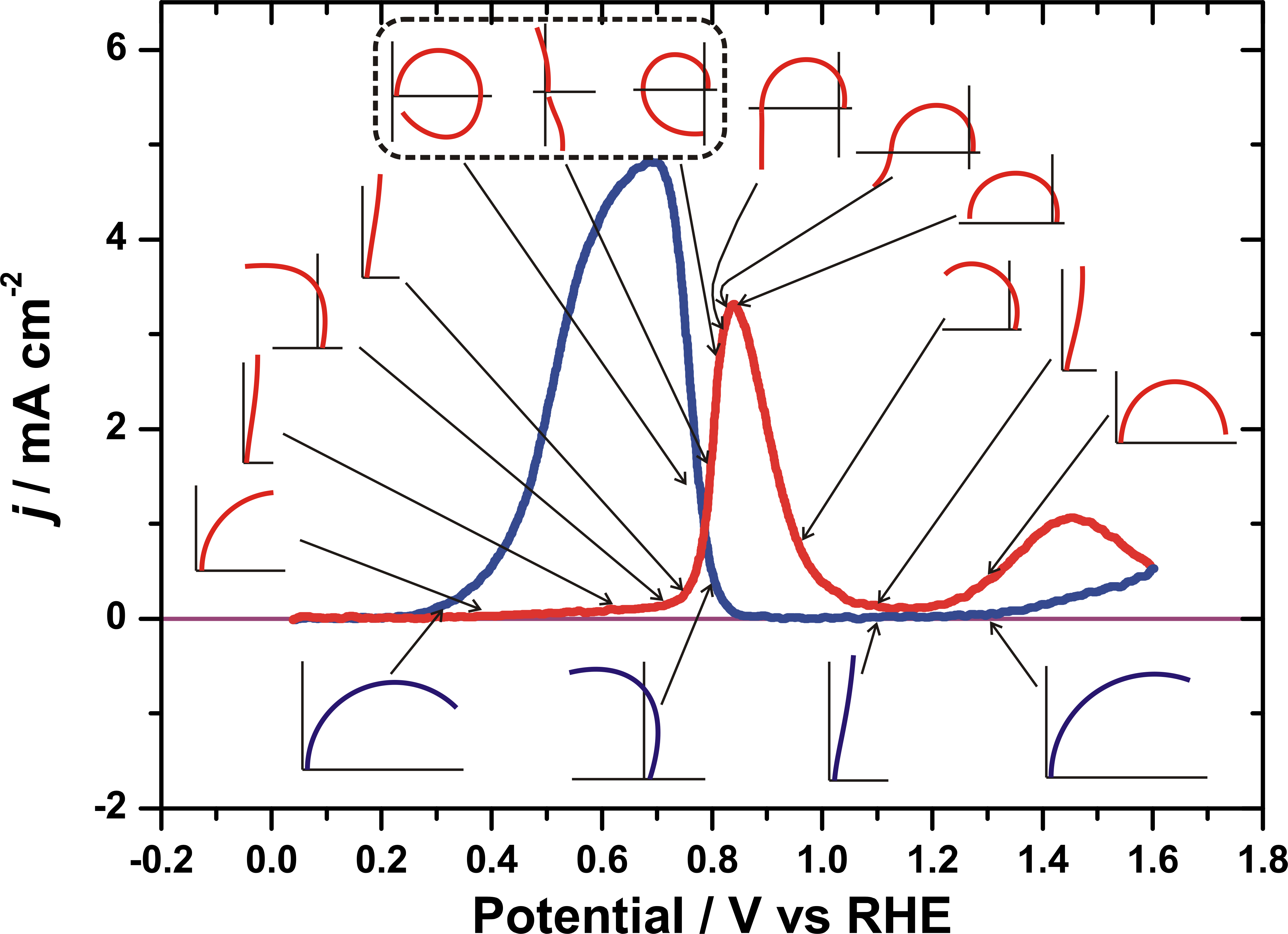
Another limitation of EIS is that there are no simple rules relating the shape of the impedance spectra or the equivalent circuit fitted to the number of species or reactions involved in a multistep mechanism. We have developed a theoretical framework for understanding the impedance of multistep mechanisms in terms of the number of species, elements and independent reactions. The maximum number of bends in a Bode plot of impedance can be related to these quantities. We have also been able to establish some rules about which types of reaction mechanisms give equivalent circuits with only resistors and capacitors, and which give circuits with inductors.
Microfluidic Devices
A microfluidic channel with the fluid exposed to different electrodes along the channel can act as an analytical device somewhat similar to the rotating ring-disk electrode. The first electrode is like the disk of the RRDE, and carries out the electrochemistry of interest, e.g., oxidation of ethanol. The second electrode is like the ring of the RRDE and can be used to detect species formed at the first electrode. The flow rate plays the role of the rotation rate in the RRDE. However, microfluidic devices offer some advantages such as high collection efficiencies and the possibility of multiple downstream electrodes. We are currently applying these devices to unravel the reaction mechanisms of oxidation of fuels such as ethanol.
Ultra-high Vacuum Surface Science Methods
We use modern surface science methods to probe the atomic-level details of the electrode/electrolyte interface, after contamination-free transfer to ultra-high vacuum (UHV). Our UHV system has (1) Low Energy Electron Diffraction (LEED), the 2-D analog of X-ray crystallography - LEED patterns give us the size and shape of the surface unit cell, (2) Auger Electron Spectroscopy (AES) which gives the types and numbers of the atoms of the surface region, (3) Thermal Desorption Spectroscopy (TDS) (using mass spectroscopy) which gives information on surface concentrations and estimates of the surface bond strengths, (4) Work Function (WF) measurements, which we use to estimate dipole moments and orientation of adsorbed molecules, and (5) Electron Stimulated Desorption Ion Angular Distribution (ESDIAD), which gives information about orientations and motions of adsorbed molecules.
This area of our research is winding down. The apparatus has allowed us to do two types of experiments relevant to electrochemistry. In the first, a cleaned and characterized single-crystal surface is taken from UHV to an electrochemical chamber, where the pressure is raised to atmospheric with Ar, the surface is immersed in an electrolyte and then some electrochemistry is carried out. The electrolyte is then drained away, and various stages of pumping bring the surface to UHV, where the nature of the surface is investigated by the above techniques. We have applied this methodology mainly to metal underpotential deposition (UPD) systems, especially Tl and Ag UPD on iodine adlayers on Pt(111). The second type of system involves adsorption or co-adsorption of molecules from the gas phase, to mimic the surface of an electrode in solution, so-called "model double-layer" studies; here we have been interested in coadsorption systems involving iodine, water, oxygen and Cs.